At every moment, subatomic particles stream in unfathomable numbers through your body. Each second, about 100 billion neutrinos from the sun pass through your thumbnail, and you’re bathed in a rain of muons, birthed in Earth’s atmosphere. Even humble bananas emit positrons, the electron’s antimatter counterpart. A whole universe of particles exists, and we are mostly oblivious, largely because these particles are invisible.
When I first learned, as a teenager, that this untold world of particles existed, I couldn’t stop thinking about it. And when I thought about it, I could barely breathe. I was, to steal a metaphor from writer David Foster Wallace, a fish who has only just noticed she’s swimming in water. The revelation that we’re stewing in a particle soup is why I went on to study physics, and eventually, to write about it.
To truly fathom matter at its most fundamental level, people must be able to visualize this hidden world. That’s where particle detectors come in. They spot traces of the universe’s most minuscule constituents, making these intangible concepts real. What’s more, particle detectors reveal beauty: Particles leave behind graceful spirals of bubbles, flashes of light and crisp lines of sparks.
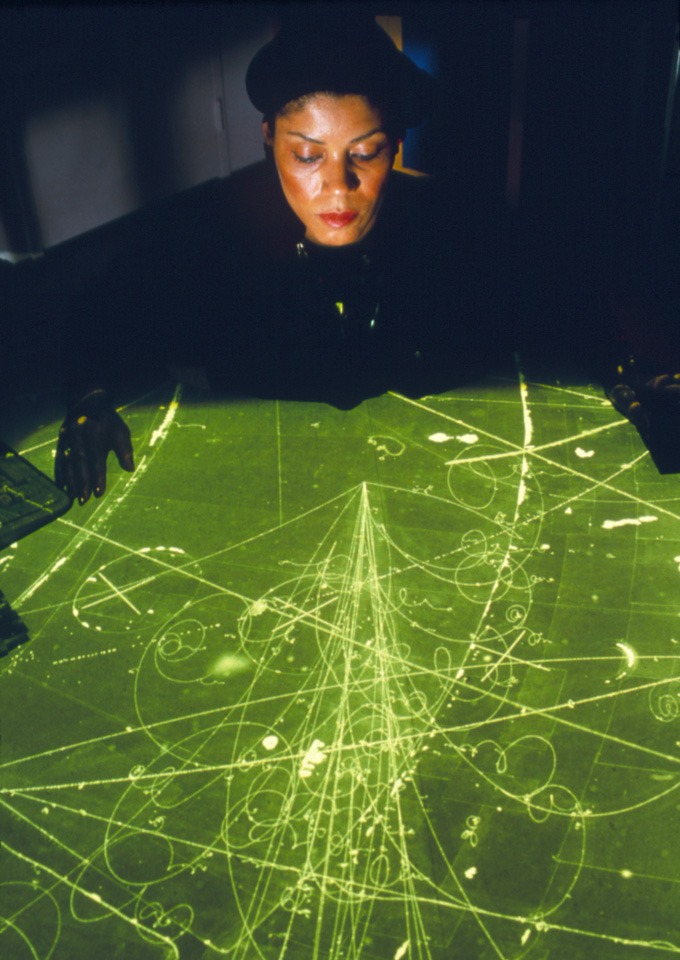
As a physics student, I spent hours examining these stunning pictures in my textbooks. I went on to build particle detectors in graduate school, and to make my own images of particles wending their way through our world.
As a particle moves through a material, it drops bread crumbs that can give away its path. Those bread crumbs come in a variety of forms: light, heat or electric charge. “Basically, every particle detector that exists is looking for one or more of those three things,” says particle physicist Jennifer Raaf of Fermilab in Batavia, Ill. Particle detectors translate the bread crumbs into signals that can be recorded and analyzed. Such signals helped reveal the physics of the standard model, a crowning achievement of science that describes the particles and forces of nature. They’re also likely to be key in the discovery of physics beyond the standard model.
As time has passed, technologies for detecting particles have vastly improved. Here are a few types of detectors that have made the invisible visible.
Through a cloud
One of the first ways scientists visualized particle tracks was with cloud chambers. Developed more than a century ago, cloud chambers are filled with a gas — often a vapor of alcohol — on the verge of condensing into liquid. When a charged particle passes through the chamber, it strips electrons from the air within, creating an electric charge that initiates condensation. A wispy line forms along the particle’s path, like a miniature contrail.
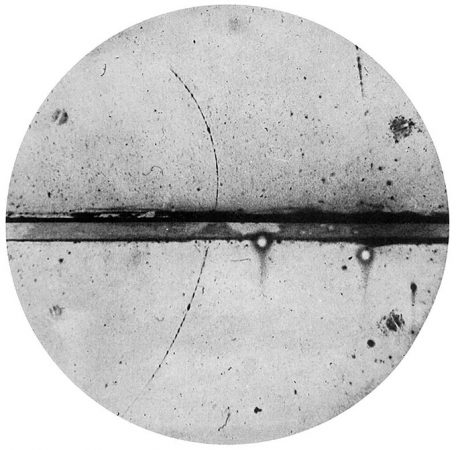
Scientists often surround cloud chambers and other detectors with a strong magnetic field, which bends particles’ paths into curves or spirals. Negatively charged particles curve in one direction, positive particles go the opposite way. Other details further characterize the particle: The amount of curvature indicates a particle’s momentum, for example.
Cloud chambers revealed a variety of previously unknown particles, including the positron and the muon, a heavy cousin of the electron, in the 1930s. These particles were mostly unexpected. At the time, physicists were barely coming to grips with the fact that particles besides electrons and protons existed.
Cloud chambers are simple enough that you can make one in your own home, using alcohol and dry ice.
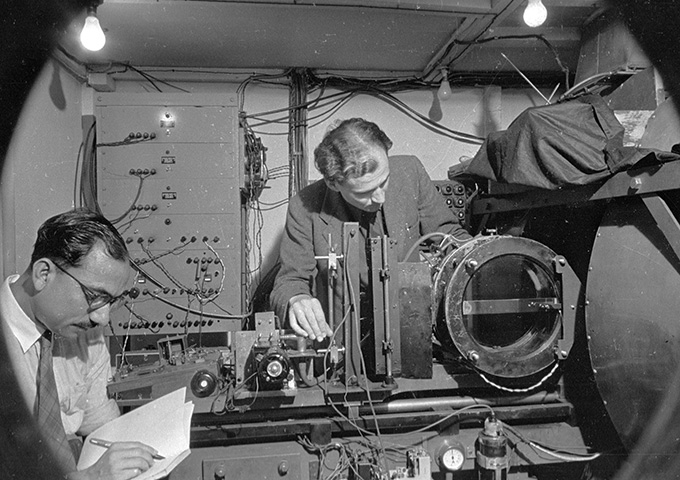
Bubble trails
The 1950s were all about bubble chambers.
When charged particles pass through liquid in a bubble chamber, they leave tiny vapor bubbles, like iridescent orbs trailing a soap bubble wand. Although the chambers are typically filled with liquid hydrogen, a variety of liquids can be used; one early prototype even used beer. Bubble chambers could be made bigger than cloud chambers, and produced sharper tracks, making it possible to observe more particles in more detail.
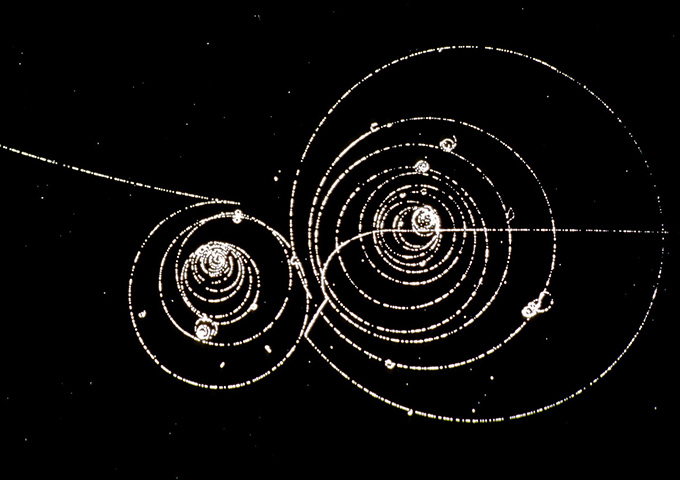
In the same decade, particle accelerators came to the fore. These accelerators produce energetic beams of particles that scientists can crash into other particles or into targets. Those collisions whip up a flurry of new particles. Scientists sent those beams into bubble chambers to watch what happened.
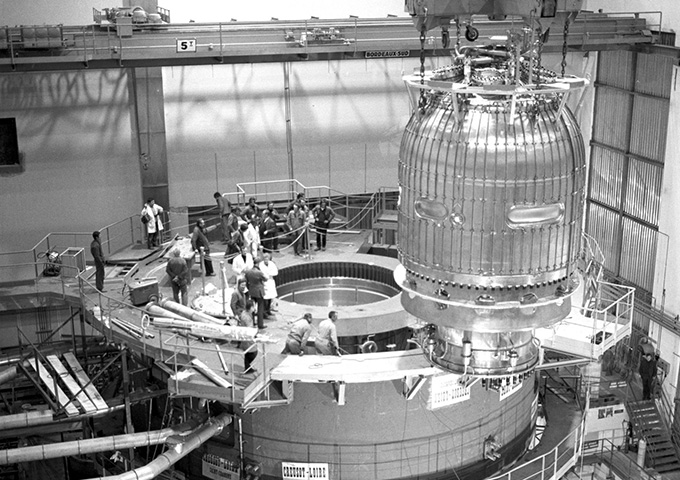
The resulting images were not only scientifically illuminating, they were stunning: If Raaf were going to get a tattoo, she says, it might be a bubble chamber image. I’ve so far resisted the temptation to get ink.
Going digital
Cloud chambers and bubble chambers had a drawback. Tracks were typically recorded with photographs, and each had to be inspected by eye for anything of interest. That process was too slow; it held physicists back from discovering the particles that might show up in only one or two out of myriad photographs, if that. To find the rarest of particles, “you can’t really be looking at pictures. You want to have that information digitized in a smart way,” says Sam Zeller, a particle physicist at Fermilab.
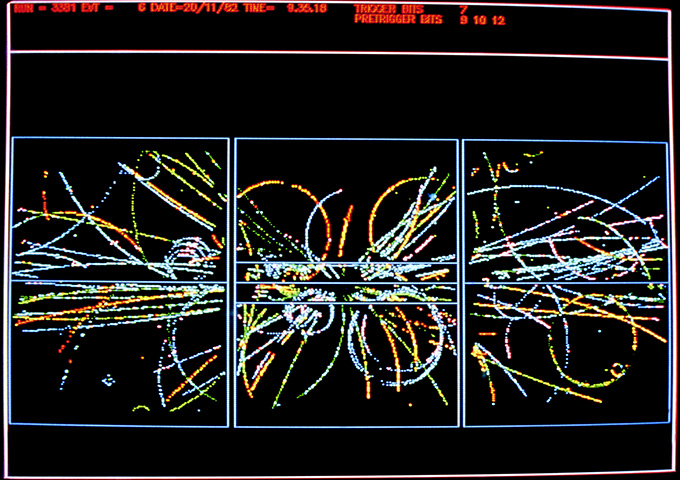
Enter the multiwire proportional chamber. Invented in 1968, this technology relies on a fine array of high-voltage wires, which record charge produced when incoming particles dislodge electrons from atoms in a gas-filled chamber. This technique could capture millions of particle tracks per second, much more than bubble chambers could achieve. And the data went directly to a computer for analysis. Multiwire proportional chambers and their descendants revolutionized particle physics, and led to discoveries of particles such as the charm quark and the gluon in the 1970s, and the W and Z bosons in the 1980s.
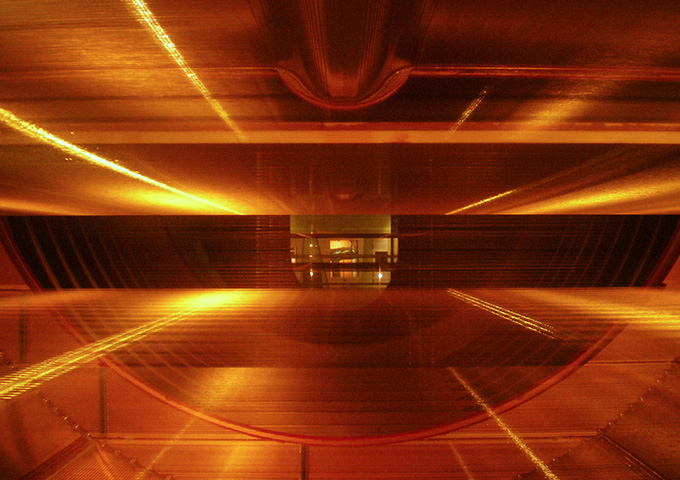
Some of the most advanced modern detectors trace their lineage back to multiwire proportional chambers, such as liquid argon time projection chambers. These detectors are high-resolution, meaning that researchers can zoom in on the details of an interaction and visualize it in 3-D. Liquid argon time projection chambers will be key to one of the biggest upcoming particle physics experiments in the United States, the Deep Underground Neutrino Experiment in South Dakota. Because neutrinos very rarely interact with matter, the experiment demands such advanced detection techniques.
Shining a light
Scientists have also devised methods to detect particles via light. When a particle moves above a certain speed limit for a given material, it emits light, known as Cherenkov light. It’s analogous to an airplane passing the sound-speed barrier and creating a sonic boom. Charged particles can also emit light when passing through materials laced with certain chemicals, called scintillators.
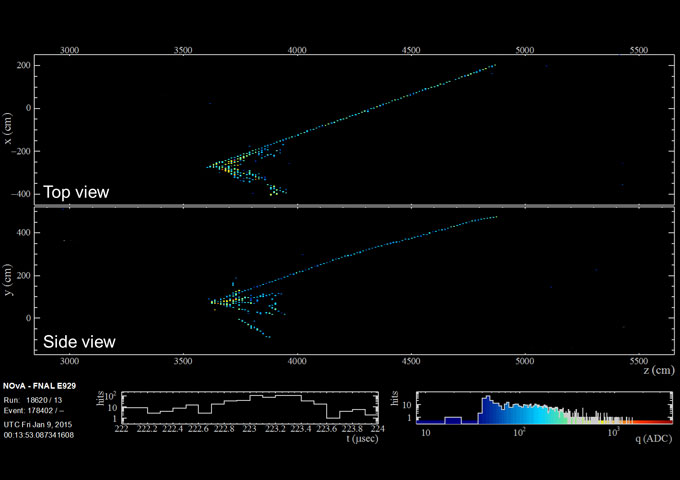
To spot the small amounts of light left behind by individual particles, scientists use photomultiplier tubes, originally invented in the 1930s, which convert light into electrical signals. These tubes could be used to pick up either Cherenkov light or scintillator light.
Scintillator detectors began to prove their worth in 1956 when a tank of liquid scintillator was used to discover the neutrino — once thought to be entirely undetectable. Liquid scintillator detectors are still common — used in the NOvA neutrino experiment at Fermilab, for example — as are detectors made of solid plastic strips with scintillator mixed in.
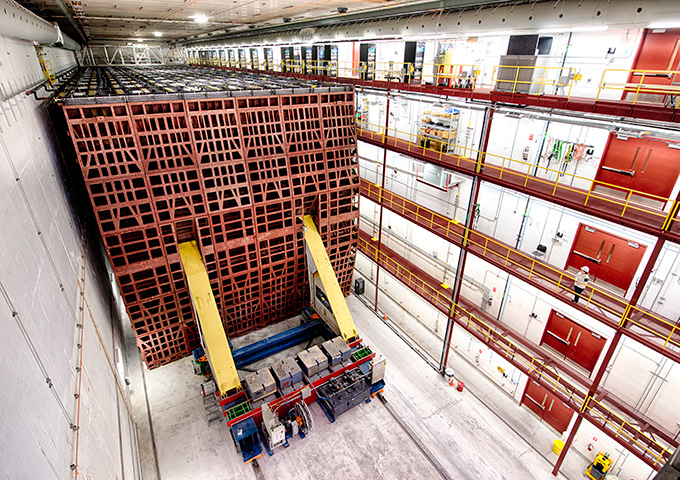
Putting it all together
Modern detectors at the world’s major particle colliders, like the detectors at the Large Hadron Collider at CERN near Geneva, throw in a bit of everything. “It’s this onion of different types of detectors; every layer is a different thing,” Raaf says.
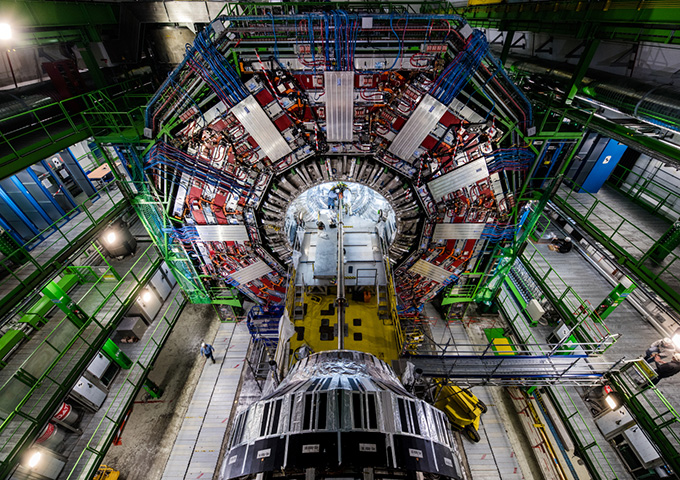
Standing multiple stories tall, these massive machines include an assortment of technologies — plastic scintillator detectors, Cherenkov detectors, descendants of multiwire proportional chambers. They also typically include detectors made from silicon that can precisely measure particle tracks based on small electric currents produced when particle pass through. These detectors all work in concert within a very strong magnet. After particles collide at the center of the detector, computers crunch the data from all the parts and reconstruct what happened in the collision, tracing out the paths the particles took.
No matter the technique, the mesmerizing subatomic hieroglyphs allow physicists to decipher the native language of matter, unveiling its constituents and the forces by which they communicate. “It’s pretty amazing that you can see the invisible,” says Zeller.
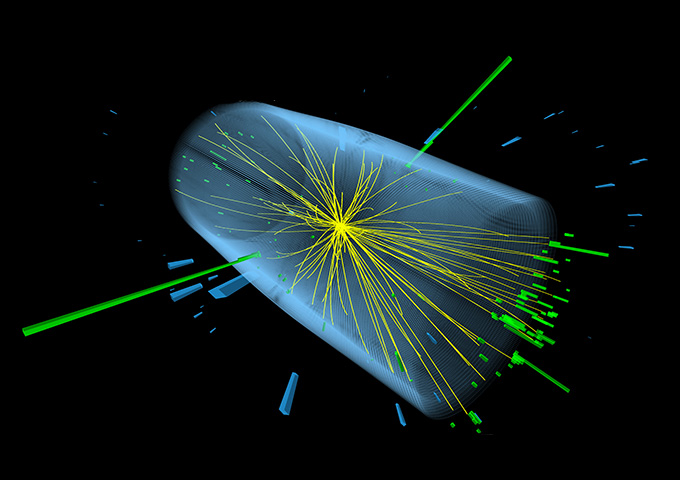